There are a number of reasons why intra-procedure CMR is an attractive option for guiding future electrophysiology procedures. First, CMR offers a number of ablation lesion imaging techniques. In addition, the ability to obtain images in arbitrary orientations opens the potential for high-quality visualization of catheters, anatomy, and electrode tissue contact. Further, the position errors introduced by registering catheter position to pre-acquired 3-D images can be largely avoided because both real-time CMR images and 3-D CMR images are acquired in the same co-ordinate system and 3-D images can be reacquired during the procedure if needed.
Over the last 15 years the basic techniques to enable fully MR-guided electrophysiology (EP) procedures have been developed. Lardo and colleagues introduced the potential of CMR for guiding EP procedures in 2000.38 Continuous MR imaging was used to guide non-ferromagnetic EP catheter positioning from an internal jugular vein to selected locations in the right atrium and right ventricle. They also demonstrated the ability to perform and monitor ablations in the MRI scanner (Figure 3A, next page). Delivery of RF ablation energy during imaging can cause significant image degradation, but this noise was dramatically suppressed by 10 MHz cut-off low-pass filtering of the ablation source. After ablation, imaging at the catheter location showed the lesion position and extent using both T2-weighted and gadolinium-enhanced T1-weighted imaging techniques.38 The onset of T2 changes at the ablation site was rapid enough that it could be used for lesion monitoring shortly after ablation (Figure 3B).
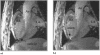 | Figure 3Example of MRI visualization of an ablation catheter positioned at the right ventricle (RV) apex (a) before and (b) after radiofrequency ablation. Post-ablation images were obtained after peripheral injection of gadolinium contrast. Figure included with (more ...) |
Subsequent work in our lab has demonstrated the ability to use real-time CMR to perform basic diagnostic EP studies.39 Imaging was performed using an unmodified clinical scanner with interactive scan plane manipulation software to guide non-ferromagnetic catheters to standard electrogram recording sites including the high right atrium, His bundle, and right ventricular apex. Electrical interference from gradient switching was adequately suppressed by 30 Hz to 300 Hz band-pass filtering such that even the low-voltage signal from the His bundle could be identified (Figure 4). Importantly, the study demonstrated that MR-guided electrophysiology measurements could be performed safely in human subjects. The topic of device safety in the CMR environment is discussed further below.
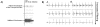 | Figure 4A: Example of the bipolar intracardiac electrograms during scanning before filtering (bottom trace) and after filtering (top trace). B: Example of bipolar intracardiac electrograms at various locations in the heart outside the scanner (left column) and (more ...) |
Other techniques relevant to EP procedures have also been performed using real-time CMR guidance. Trans-septal catheterization is required for left atrial catheter ablation. While generally safe, the procedure can be difficult in the setting of distorted atrial anatomy and carries the risk of serious complications such as aortic puncture. The ability of real-time CMR to guide trans-septal punctures under direct visualization of the needle, atria, fossa ovalis, and surrounding vasculature has been nicely demonstrated.40,41 Retrograde catheterization of the left ventricle from the femoral artery is commonly required for VT ablation and has also been performed under real-time CMR guidance.42
Recent advances promise to take MR-guided EP from these initial feasibility studies to safe, efficient practice. These advances include 1) intra-procedure ablation lesion monitoring techniques, 2) faster 2-D and 3-D imaging, 3) improved device visualization, 4) intuitive 3-D anatomy and intracardiac electrogram visualization, and 5) MRI-safe device construction techniques.
INTRA-PROCEDURE LESION MONITORING
Perhaps the most significant advantage of CMR-guided ablation therapy is the potential to visualize ablation lesions with high spatial and temporal resolution. The typical end-point of current ablation procedures is absence of electrical conduction across the ablated region and/or an in-ability to reinduce the clinical arrhythmia with cardiac pacing and medications. However, propagation of electrical signals through the heart is affected by a number of factors including the tissue temperature change induced by ablation.
43,44 Some of these factors may be reversible over time leading to arrhythmia recurrence.
35,36 As described below, CMR appears capable of delineating areas of permanent tissue damage caused by ablation. Using CMR lesion imaging to guide ablation could improve the procedure end-point from assessment of potentially transient electrophysiologic changes to a more direct assessment of complete lines of permanently damaged tissue in the region of interest.
A 500 kHz radiofrequency (RF) current is the most commonly used ablation source used for electrophysiology procedures. Cryothermy, ultrasound, laser, and microwave ablation are also being investigated. Ablation lesions can be visualized because CMR is able to detect specific changes in proton precession and relaxation properties resulting from heating and heat-induced biophysical changes in cardiac tissue including interstitial edema, hyperemia, protein conformational changes, cellular shrinkage, and tissue coagulation.38 Acute interstitial edema is likely responsible for the hyperintense region corresponding to the area of acute RF ablation damage observed by T2-weighted fast spin echo imaging38,45 (Figure 5). Dickfield and colleagues found that this hyperintense region correlated well with necrotic lesion size on gross pathology and also noted that gaps between lesions on imaging corresponded with lesion gaps on pathology.46 Lesion visualization by T2-weighted imaging has been reported as soon as 2 minutes after ablation, and stable imaging characteristics have been observed from 30 minutes to 12 hours post-ablation.38,46 This could make T2-weighted MRI a tool to evaluate lesions and lesion continuity over the course of an ablation procedure.
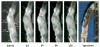 | Figure 5Example of non-contrast T2-weighted MR imaging of right ventricular epicardial RF ablation lesions with pathologic correlation. Stability of the imaged lesion size is demonstrated from 30 minutes to 12 hours after ablation. Figure included with permission (more ...) |
T1-weighted non-contrast-enhanced MR imaging of RF ablation lesions has also been investigated.46 T1 changes may be related to tissue heating, protein denaturation, and changes in intracellular and extracellular free water distribution during and following ablation.45–47 Similar stability of imaging characteristics were reported from 30 minutes to 12 hours following ablation, though the lesion contrast by T1-weighted imaging appears to be less than for T2-weighted imaging.46
Gadolinium delayed enhancement CMR (DECMR) can provide better visualization of RF ablation lesions compared with non-contrast imaging techniques (Figure 6). The time to achieve full enhancement of RF ablation lesions, 1 to 2 hours, is considerably longer than for DECMR of myocardial infarct scar.48 However, good correlation with pathologic lesion size was noted for intermediate enhancement patterns from 1 minute to 2 hours after contrast injection, allowing lesion extent to be assessed without waiting for full enhancement.48 The 1 to 2 hour interval required for renal clearance between repeated dosing of gadolinium and the ceiling on total allowable gadolinium dose limit the use of this technique for serial lesion assessment during a procedure.45 Still, gadolinium-enhanced imaging may be useful for evaluating gaps in ablation lines after completion of a procedure to assess the need to place additional lesions.
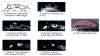 | Figure 6Example of gadolinium-enhanced T1-weighted MR imaging of right ventricular epicardial RF ablation lesions with pathologic correlation. Different lesion enhancement patterns are seen from 1 minute to 2 hours after contrast injection. Figure included with (more ...) |
Other methods for monitoring ablation lesion formation during RF energy application are also being investigated. Proton resonance shift thermography is an MRI technique that takes advantage of the decrease in the proton resonance frequency with increasing temperature.49 This technique has been used to follow tumor ablation in the uterus, liver, prostate, and brain using diverse energy sources including RF, high-frequency ultrasound, laser, and microwave.50–55 Its use for following RF ablation in the beating heart is being investigated. Current-vector mapping has also been described for monitoring the extent of tissue power deposition during RF ablation.56
While most cardiac ablation lesion MRI studies have been performed in roughly 10 mm thick ventricle, imaging the less than 3 mm thick human atria is of particular clinical interest given the difficulty of achieving long-term pulmonary vein isolation following atrial fibrillation ablation. Peters et al. demonstrated 3-D DECMR of left atrial ablation lesions 10 to 15 minutes after contrast injection using image-based respiratory gating.24 This gating technique, also known as respiratory navigator imaging, allowed higher-resolution 3-D imaging to be performed without the need for prolonged breath-holding by tracking diaphragm position on fast 1-D images and collecting 3-D image data within a narrow range of diaphragm positions. Current applications have used a roughly 100 ms mid-diastolic acquisition window timed to precede atrial systole to reduce atrial motion during imaging. Image resolutions of 1.25 × 1.25 × 2.5 mm (reconstructed to 0.6 × 0.6 × 1.25 mm) have been demonstrated. McGann et al. combined this technique with 3-D visualization and quantification to assess left atrial scarring before and following atrial fibrillation ablation. They found that subjects with more than 13% left atrial delayed enhancement following ablation had a nearly 20 times higher chance of being free from atrial fibrillation than those with low amounts of post-ablation delayed enhancement.57 The technique is now being used to image immediately following the procedure to target further ablation and potentially reduce the need for repeat procedures. Interestingly, high-resolution 3-D DECMR may also be useful for better identifying patients who will respond poorly to current ablation techniques.58 Of note, such high-resolution imaging is not yet feasible in all patients. In the above studies, imaging took 5 to 10 minutes, and 10% to 30% of patients were excluded from analysis because of poor image quality that was attributed to patient motion, significant arrhythmia, or incorrect inversion time selection. Techniques to improve the speed and reliability of high-resolution CMR require further investigation.
FASTER MR IMAGING
While cardiac gating can be used to generate MR images with excellent spatial resolution by splitting data collection over multiple heart-beats, real-time CMR requires a more deliberate trade-off between temporal and spatial resolution. To visualize catheters adequately, MRI-guided EP procedures require an in-plane spatial resolution of around 2 mm
2. The target temporal resolution is 7 frames per second (fps), the usual X-ray fluoroscopy frame rate for clinical EP procedures.
Since the initial 1 fps imaging used to guide the first MR-guided EP procedure38 faster, stronger gradients have increased the temporal resolution capabilities of fast gradient-recalled echo sequences to the 5 fps range.59 Improved gradient performance and B0 field homogeneity have also allowed real-time imaging to be performed with coherent steady state pulse sequences (i.e. steady-state free precession, true fast imaging with steady-state precession (true-FISP), or Fast Imaging Employing Steady-state Acquisition). These sequences provide increased contrast-to-noise performance at a given frame rate compared with fast gradient-recalled echo sequences.59 Parallel imaging techniques can provide additional improvement in temporal resolution without sacrificing spatial resolution. These techniques accelerate imaging by covering the region of interest with multiple receive coils and using the different spatial sensitivities of these coils to correct for undersampling of image data.60 The acceleration achieved from acquiring less data is countered by the increased processing time required for estimating coil sensitivities and for performing the parallel reconstruction.61 Also, for a given number of receive coils, more undersampling leads to deteriorating image signal-to-noise or a reduced ability to suppress aliasing artifacts.62 Balancing these issues, 15 fps true-FISP cardiac imaging with 128 phase encode lines can be performed using an 8-channel receive coil array and optimized reconstruction hardware.63 Commercial MRI systems now commonly have multichannel receivers and parallel imaging options. The performance of these systems is currently in the range of what is needed to perform CMR-guided EP procedures at 5 fps with acceptable image quality.61
While the current imaging rates are adequate for a single 2-D image plane, ideal visualization of the device, target anatomy, and surrounding reference anatomy may require multiple 2-D image planes or even 3-D imaging. Other techniques that can improve imaging speed while balancing imaging quality include non-Cartesian k-space sampling, temporal data sharing between images, and adjusting the trade-off between temporal and spatial resolution.59 These techniques may be particularly useful to accelerate imaging of reference anatomy views that are not depended on for device tracking. Use of 32-channel receive arrays to perform more rapid 3-D cardiac imaging and parallel transmission techniques to permit more efficient parallel data collection are also under active investigation.63–65
DEVICE VISUALIZATION AND NAVIGATION
While fluoroscopy provides projection images where the entire catheter body and tip are easily visualized, 2-D MR images typically depict a slice through the body that is around 5–10 mm thick. Curved devices such as catheters may pass in and out of the MR imaging plane leading to mis-interpretation of the device tip position. We have noted in preclinical studies that poor delineation of the tip position can result in tissue contact trauma, such as local hemorrhage. In addition, for electrophysiology ablation procedures the device tip contains the energy source. Misestimating the tip/tissue contact region can lead to inaccurate placement of ablation lesions.
During our feasibility studies, tip location has mostly been performed using interactive real-time sequences with a user interface that permits adjustment of the scan plan during image acquisition. Part of the catheter is first identified on some imaging plane, and the plane is manually adjusted until the tip is located. For vascular procedures where the device is constrained to a co-planar segment of blood-vessel, manual plane manipulation is acceptable since only minor image plane translations are needed to visualize the device tip and relevant anatomy. For navigation in cardiac chambers where the device tip location is less constrained, the frequent need for manual plane manipulation necessitates a skilled operator for image plane manipulation and can distract from efficient procedure work flow.
One approach to this problem is to automatically direct imaging to the device location using position sensors located in the catheter. Fifteen years ago Dumolin et al. described using 1-D projection MR imaging along the x, y, and z directions to identify the 3-D position of small receiver coils located in a catheter tip.66 This position tracking technique has since been interleaved within real-time imaging sequences to automatically move the image plane position to the catheter tip location during device manipulation.61,67 While a single tracking coil is sufficient to simply shift the image position, multiple tracking points are needed to maximize visualization of the device body or to orient imaging relative to the catheter tip direction. Multicoil designs and tracking algorithms have been developed to reduce the need for separate matching circuits in space-constrained catheter lumens.68,69 Other magnetic field and electric field-based position finding techniques have been developed for medical device tracking that could also be used for catheter tracking in the MRI scanner.70–72 Some of these systems generate both position and orientation information for each sensor assembly.72,73 These systems may provide more accurate and catheter space-efficient options for device tracking. They can also reduce the performance penalty and avoid the scanner-specific complexities associated with interleaving tracking and real-time imaging sequences.
An alternative technique for device visualization uses non-slice-selective imaging to produce an effect similar to projection X-ray fluoroscopy.74,75 The non-slice-selective catheter imaging plane can be intersected with slice-selective images containing the target anatomy to assist guidance of the catheter tip. This technique may be used to provide a “fluoroscopy” view of devices using a number of catheter antenna designs 69,76–78 (Figure 3A).
Another factor that affects device navigation in the MRI environment is the physical constraint of performing procedures near and within the narrow MRI bore. The availability of shorter, wider-bore, high-field MRI scanners is making this less of an issue. Remote catheter steering is also gaining interest in the EP field to facilitate point-by-point electrical mapping of the cardiac chambers and assist stable device placement during ablation.79,80 Some of these techniques may be amenable to use in the MRI environment. A robotic catheter manipulation system that uses steerable sheaths with multiple pull wires was recently used to perform atrial fibrillation ablation in patients.81 A magnetic remote steering technique has also been described that utilizes the torque generated by current-carrying coils in the static MRI magnetic field to deflect a catheter tip.82,83
3-D ANATOMY AND INTRACARDIAC ELECTROGRAM VISUALIZATION
The ability to generate real-time images with arbitrary orientations in addition to anatomically detailed 3-D images with flexible tissue contrast makes CMR well suited for navigating complex arrhythmia anatomy and delineating complex ablation patterns. This flexibility also introduces the potential for disorientation and information overload. Appropriate displays and user-interfaces tailored to the work flow of an EP procedure are needed to manage this flexibility. Because thin-slice real-time imaging can intersect anatomy in unfamiliar ways, 3-D visualizations that plot real-time images oriented relative to reference images can be helpful (
Figure 7). A basic imaging interface for MR-guided EP procedures would provide a convenient way to “book-mark” and access reference cardiac views, switch between real-time and lesion visualization sequences during the procedure, appropriately present lesion images for ablation line continuity assessment, and display the relationship of stored images, catheter position, and intracardiac electrogram characteristics in 3-D.
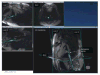 | Figure 7Example of using automatic catheter highlighting and reference image planes to navigate complex 3-D anatomy using real-time MRI. The anatomic location of the catheter position on the image labeled LAX2 is better appreciated when overlaid with long and (more ...) |
INTERVENTIONAL MRI DEVICE SAFETY
The most important consideration for any new diagnostic or therapeutic approach is safety. While a number of studies have been performed to determine the safety of conventional MRI with regard to electromagnetic energy exposure and tissue heating,
84,85 interventional procedures add additional considerations and raise new safety concerns.
86–89 The most straightforward aspect to MRI device safety is the avoidance of ferromagnetic materials that could experience significant forces when brought close to the scanner. Though MRI-unsafe objects, such as ferromagnetic scissors and needle drivers, may be needed during the preparatory phase of a procedure, until MRI-compatible alternatives are available a system must be in place to methodically track and remove such objects before approaching the scanner. The electric current associated with defibrillation can also lead to strong displacement forces in high magnetic fields and should be performed with the defibrillator pads maintained a safe distance from the scanner bore.
90 Similar attention is needed to address the ferromagnetic properties and MRI electromagnetic interference compatibility of other equipment associated with electrophysiology procedures including physiology monitoring equipment, ablation and pacing sources, and anesthesia apparatus. Clear marking of high-field areas and secure placement of objects that may experience magnetic forces is mandatory so that appropriate pieces of equipment are kept at a safe distance.
91
An additional safety concern particular to CMR-guided cardiovascular procedures is the significant heating that can result from RF transmission-induced current in extended metallic objects such as guide-wires, wired electrodes, and metal-braided catheters.87,88 This induction is more pronounced when portions of the device are located close to the RF transmit body coil housed within the edge of the scanner bore. Device length is an important factor in efficient coupling and heating; however, many other parameters can influence the sudden onset of significant heating in the setting of an interventional procedure.88,92 The simplest way to avoid this problem is to construct devices from non-metallic components when possible. Polymer materials for catheter braiding, such as Dacron and Kevlar, and composite materials for guide-wires, such as glass-fiber-reinforced plastics, can be used to achieve device functional characteristics such as torqueability, stiffness, and kink resistance.38,93 Several approaches have also been developed to avoid significant induction heating in structures that require conductivity. Wires made from high-resistance alloys and gold-sputtered thread have been used to obtain intracardiac electrograms during imaging, with significant reduction of electrode heating.94 For structures where efficient power transfer is required, such as pacing or ablation electrodes, high-frequency RF chokes can allow passage of signal lower than a few MHz while blocking unwanted MR transmit frequency currents.95,96 A promising heating suppression technique for position tracking and intravascular imaging coils that need to pass differential mode signals at the same frequencies as unwanted common mode induced currents is to place thin transmission line transformers in the signal-carrying cables.77,97,98 Other strategies such as detuning and decoupling of circuits prone to heating,78,99 fiber-optic transmission of signals,100 and use of inductively coupled resonators for wireless device tracking101 are also considerations for new device design.25,90 Using surface coils instead of the higher-power body coil for RF transmission has also been proposed as a way to reduce RF current induction in devices.102 Now that academic sites and imaging companies are focusing on heating-safe device development, more rapid progress in this area is expected.
Transitioning proof of concept studies to clinical electrophysiology procedures requires collaboration between academic centers, imaging and device companies, and regulatory agencies. The first CMR-guided electrophysiology procedure in a patient was performed using custom catheters made to clinical specifications by a clinical catheter manufacturer.39 Prior to use in a human an investigational device exemption was obtained from the Food and Drug Administration (FDA). As part of this device exemption, catheter heating and reduction of heating using RF filtering was demonstrated in a series of device positions and orientations within the scanner using high specific absorption rate (SAR)-imaging protocols.39 In addition, safe use of the catheter in animals was documented prior to human studies. Though catheters with embedded imaging coils provided improved device visualization in the animal studies, these devices were not approved for or used in patients. Before generally applying CMR guidance to interventional electrophysiology, more standardized MRI-compatible catheter safety guidelines and device testing protocols need to be developed.
The compatibility of MRI with implanted devices such as pace-makers and implantable cardiac defibrillators (ICDs) is also an important consideration in performing interventional MRI studies in the electrophysiology patient population. Particular concerns include static magnetic field-induced movement of the device and scanning-induced programming changes, device inhibition, activation of tachyarrhythmia therapies, and lead currents leading to heating or cardiac stimulation.103–106 Modern devices address some of these concerns with the use of less ferromagnetic material and improved resistance to electromagnetic interference.107 A number of devices have been carefully studied during in-vitro and animal MR imaging, and experience is growing for safe cardiac MRI scanning in patients with selected devices under controlled scanning conditions.25,107,108 This experience includes use of sequences relevant to modern CMR with SAR characteristics similar to those used for real-time MRI.25 Still, the number of patients and devices studied thus far is limited, and further work is needed to develop manufacturer protocols for establishing conditional MRI safety of pace-makers and ICDs. Carefully designed protocols for patient selection, monitoring, and scanning also need to be developed before imaging of patients with devices can be more routinely performed. The impact of device-related artifacts on cardiac image interpretation also needs to be more carefully studied.