For decades radiographs were the only form of medical imaging, but human curiosity and the insatiable appetite to improve medical imaging grew. New tools were rolled out, and two-dimensional flat images gave way to three-dimensional imaging, four-dimensional imaging (three-dimensional imaging in real time), functional imaging, and molecular imaging.
Computed Tomography
In the beginning of the twentieth century, Alessandro Vallebona, an Italian radiologist, invented conventional tomography. Conventional tomography is created by simultaneously moving the X-ray source and X-ray detector in tandem so as to keep an object of interest in the center point of the scan plane and blur out all other objects. Conventional tomography evolved, but it was still ineffective for imaging soft tissues and large areas of the body.
7
Seventy-six years after the birth of medical imaging, computed tomography (CT) was developed using radiographic projections from multiple angles and then building a two-dimensional image with a mathematical model that incorporated all of the projected data. In 1967, Sir Godfrey Hounsfield invented the first CT scanner at EMI research laboratories. The first live patient was scanned on October 1, 1971. Alan M. Cormack (who created the early mathematical models used in CT) and Hounsfield jointly received a Nobel Prize in 1979 for development of “computer assisted tomography.”4
Computed tomography is one of most important medical innovations in human history. Images display soft tissue contrasted with anatomic detail, facilitating unprecedented diagnostic accuracy. Two-dimensional, cross-sectional images are created that can be arranged in space to generate a volume. By the early 1980s, more than three million CT studies had been performed. Presently that number has grown to well over 100 million CT studies annually,8 with CT becoming the modern doctor’s “truth machine.”
Many technological advancements were made in CT, with improvements in scan speed, smaller slice thickness, decreased radiation dose, and better image quality. Twenty-five years ago, a typical CT study could take dozens of minutes depending on the scanned volume and exact machine, whereas nowadays CT studies can be performed in a fraction of a second, covering large areas of the body, even using more than one scan energy. The CT slices in modern machines can be as thin as fractions of a millimeter.7 New image reconstruction techniques and more efficient, larger detector materials have decreased radiation dosages by more than half,9 while continuously improving image quality.
New applications, such as CT perfusion for detection and quantification of cerebral stroke, soon followed. Recently, the therapeutic window (i.e. the timeframe within which treatment can be initiated as measured from the onset of symptoms) significantly increased thanks to the accuracy of perfusion CT, affording many more patients the chance for effective embolectomy. If CT perfusion reveals a sufficient penumbra, neuro-interventional therapy may be attempted up to 24 hours after the onset of symptoms.10 Together with CT angiography, this new approach has revolutionized the world of stroke therapy (Figure 1).
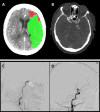 | Figure 1 CT Brain Perfusion, CTA, Brain Angiography |
Another frontier is cardiac CT with coronary CT angiography (CTA). In the setting of low- to moderate-risk chest pain, coronary CTA is a non-invasive method of evaluation with high sensitivity and specificity.11
Future directions include radiation dose reduction and spectral (multi-energy) CT. Spectral CT uses a single acquisition performed at multiple energies to extract more information about tissue differentiation based on the way different photon energies are absorbed in different tissues. Clearly, CT will continue to bring new insights into human diseases.
Magnetic Resonance Imaging
The history of the development of magnetic resonance imaging (MRI) is complex and involves many contributions from clinical, scientific, and technical fields. Two scientists, Felix Bloch and Edward Purcell, simultaneously and independently developed the concept of nuclear magnetic resonance (NMR), for which they were jointly awarded the Nobel Prize in Physics in 1952.
4 In 1973, Paul Lauterbur published the first NMR images,
12 and he later received the 2003 Nobel Prize in Physiology and Medicine.
4
Magnetic resonance imaging is based on different physical principles than CT. A powerful magnet is used to produce a very strong fixed magnetic field around the patient, and radiofrequency pulses are used to excite protons within the body. As the excited protons relax back to a resting state, they return signals that are captured and mapped into an image.
Today, MRI is used in virtually every medical subspecialty. Its superior soft tissue contrast and anatomic detail make it the crown jewel of medical imaging. It has become essential in oncology, where scanning is routinely utilized for preoperative staging and to determine the extent of disease throughout the body. Whole-body MRI is now a reality, especially when combined with functional nuclear medicine imaging, i.e. positron emission tomogramphy MRI (PET-MRI); the surface has barely been scratched for what is possible via this modality.
Cardiac MRI is increasingly used for conditions such as cardiomyopathy or acute myocardial infarction. Not only morphology, but also cardiac function can be assessed with cine images of ventricular wall motion, valvular function, and T1 mapping of cardiac muscle tissue fibrosis.13–15
Functional MRI (fMRI) is used in clinical imaging of central nervous system diseases (e.g. vascular mapping for diagnosis and grading of gliomas) (Figure 2).15 Because fMRI measures brain activity by detecting changes associated with blood flow, it can be used in surgical planning. Robust research initiatives with fMRI and diffusion tensor imaging to study brain and spine nerve fiber tracts are underway.16,17 Magnetic resonance spectroscopy is used to measure the relative concentration of different endogenous metabolites in tissues.18
Ultrasound
Ultrasound (US) is not one technology but many, with an intricate history involving many brilliant scientists and physicians since its inception in the twentieth century. The backstory of diagnostic ultrasonography began with the study of bats in the late 1700s by Lazzaro Spallanzani. Spallanzani tried to understand how bats fly at night and hypothesized that bats relied on sound to navigate.
19 In 1826, Jean-Daniel Colladon calculated the speed of sound in water estimating close to the currently known value of 1,482 m/s.
20 The Doppler effect, first postulated in 1842 by Christian Doppler, is an essential part of almost every US study and forms the basis of US evaluation of blood flow. Next the Curie brothers discovered piezoelectricity. Piezoelectric crystals vibrate when subjected to an alternating current. This is the basis for the development of US transducers.
21
Interestingly the use of US in medicine started with therapeutic applications, not with imaging. The potentially destructive power of a focused US beam was recognized as a valuable therapeutic tool in the second decade of the twentieth century as a surrogate for neurosurgery, rehabilitation, and treatment of rheumatoid arthritis. In the 1940s, US for medical imaging was first investigated by a physician named Karl Theo Dussik. Dussik attempted to locate brain tumors and identify the cerebral ventricles. His initial experiments were reported in 1942.20,22 In 1958, Ian Donald, a gynecologist, first used US to study the unborn fetus, uterus, and pelvis.23
Ultrasound uses high-frequency sound waves, above the range of human hearing, which are transmitted into the body. The echoes reflected are mapped into an image based on the amplitude and time delay of the returned signals. This modality has advantages over CT. Because US has no ionizing radiation, is inexpensive, is widely available, and is performed face-to-face with patients, it is particularly important in pediatric imaging. Although conventional US is operator-dependent, new three-dimensional US is less so.
A significant US advance was the development of US contrast materials comprising microbubbles that enhance the echo signal reflected from the imaged tissue. Contrast-enhanced US (CEUS) not only improves direct visualization of blood vessels, but also permits tissue characterization in solid organs. Using dynamic enhanced scans, lesion perfusion combined with the superior resolution of modern US can reveal microvasculature and may help unlock the mysteries of tumor angiogenesis in micron-sized abnormal blood vessels (Figure 3).24
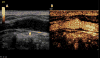 | Figure 3 Contrast-enhanced Ultrasound (CEUS) |
Ultrasound elastography, a relatively new technique, provides semi-quantitative and quantitative measurements of tissue stiffness. Because tumor tends to be stiffer than surrounding normal tissue, elastography can assist with both lesion detection and characterization, and has been used in breast, thyroid, and liver imaging.
Another promising development is US fusion, where other modalities such as CT or MRI are combined with US. The different scans are spatially co-registered using the patient’s own anatomic landmarks. During real-time scanning, the US transducer can be moved using electromagnetic sensors and a field generator that enables comparison of lesions depicted on several modalities. It also permits navigation using real-time US for focal therapy such as for prostate cancer, based on the previously detected lesions in other modalities (Figure 4).25,26
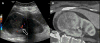 | Figure 4 Ultrasound Computed Tomography (US-CT) Fusion |
Nuclear Imaging and Hybrid Scanners
Nuclear medicine uses radioactive tracers to create functional imaging. Unique sensors detect the radioactive emission of these tracers. Single-photon emission computed tomography (SPECT) and positron emission tomography (PET) both use co-registration of a morphological scan (commonly CT) with a functional nuclear medicine scan.
The SPECT scans measure gamma rays, while PET scans use a tracer that accumulates in body tissues and is metabolized, after which positrons are emitted. When positrons encounter electrons, they annihilate each other, emitting a pair of gamma rays simultaneously. Special detectors register the simultaneous arrival of the pair of gamma rays and the number of such arrivals. The data are used to map metabolic activity in the organs of the body. James Robertson built the first single-plane PET scanner at the Brookhaven National Laboratory in 1961.
Functional and morphological co-registration (i.e. the first hybrid design) was first proposed for a PET-CT scanner by David Townsend and Ronald Nutt. A prototype hybrid clinical PET-CT scanner in a single gantry was first used clinically in 1998.27 Functional metabolic imaging is now routinely combined with high spatial resolution scans in clinical practice using not only PET-CT but also PET-MRI, and more.
Interventional Medical Imaging
One important early development in interventional imaging was catheter angiography. In 1927, Egas Moniz, a neurologist from Portugal, performed the first cerebral angiography, for which he was awarded the Nobel Prize in Medicine in 1949.
28 In 1929, the first cardiac angiography was performed by Werner Forssmann in Germany when he inserted a catheter into his own arm vein and passed a catheter into his heart. He shared the 1956 Nobel Prize in Medicine with Andre F. Cournand and Dickinson W. Richards for pioneering cardiac catheterization.
29 Recent breakthroughs in catheter angiography for diagnosis and treatment of cardiovascular emergencies include removal of brain clot using new generations of retrievable stents, occlusion of active arterial hemorrhage in trauma patients, and in selective tumor ablation.
Other image-guided therapies have rapidly gone from the bench to the bedside in the last two decades. Image-guided biopsy is used daily in almost every organ including thyroid, lung, liver, breast, and bone. Minimally invasive procedures for abscess drainage and directed tissue ablation using real-time imaging guidance are in common practice. Tissue ablation may be accomplished using radiofrequency ablation, cryoablation, or stereotactic laser ablation.
Another example, high-intensity focused ultrasound (HIFU), appeared in the early history of US. First proposed by Lynn et al. in 1942,20,21 HIFU guided by real-time imaging uses an external beam of therapeutic US to focally heat and ablate targeted tissues without damaging the surrounding normal tissue. The result of HIFU is irreversible coagulation necrosis and mechanical damage through cavitation. For example, MR-guided focused ultrasound (MRGFUS) has been successfully used to treat medication-resistant Parkinson’s disease.30–32 This technology is also used for many other conditions and locations ranging from movement disorders requiring ablation of deep brain nuclei (Figure 5), to ablation of uterine fibroids, prostate cancer, and bone metastases.
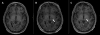 | Figure 5 Magnetic Resonance-guided Focused Ultrasound (MRGFUS) |