| |
|
RMMJ
Rambam Maimonides Medical Journal
Rambam Health Care Campus 2015 July; 6(3): e0025.
ISSN: 2076-9172 Published online 2015 July 30. doi: 10.5041/RMMJ.10210
Special Fifth Anniversary Issue
Lung Edema Clearance: Relevance to Patients with Lung Injury Zaher S. Azzam, M.D.1 and Jacob I. Sznajder, M.D.2* 1Internal Medicine “B”, Rambam Health Care Campus, Department of Physiology and Biophysics, The Rappaport Family Faculty of Medicine and Research Institute, Technion, Israel Institute of Technology, Haifa, Israel 2Division of Pulmonary and Critical Care Medicine, Northwestern University, Chicago, IL, USA.
|
Pulmonary edema clearance is necessary for patients with lung injury to recover and survive. The mechanisms regulating edema clearance from the lungs are distinct from the factors contributing edema formation during injury. Edema clearance is effected via vectorial transport of Na+ out of the airspaces which generates an osmotic gradient causing water to follow the gradient out of the cells. This Na+ transport across the alveolar epithelium is mostly effected via apical Na+ and chloride channels and basolateral Na,K-ATPase. The Na,K-ATPase pumps Na+ out of the cell and K+ into the cell against their respective gradients in an ATP-consuming reaction. Two mechanisms contribute to the regulation of the Na,K-ATPase activity:recruitment of its subunits from intracellular compartments into the basolateral membrane, and transcriptional/translational regulation. Na,K-ATPase activity and edema clearance are increased by catecholamines, aldosterone, vasopressin, overexpression of the pump genes, and others. During lung injury, mechanisms regulating edema clearance are inhibited by yet unclear pathways. Better understanding of the mechanisms that regulate pulmonary edema clearance may lead to therapeutic interventions that counterbalance the inhibition of edema clearance during lung injury and improve the lungs’ ability to clear fluid, which is crucial for patient survival.
Keywords: Acute lung injury, alveolar epithelium, alveolar fluid clearance, Na,K-ATPase, pulmonary edema |
Pulmonary edema is a life-threatening condition of fluid excess in the lungs that causes impaired gas exchange with consequent symptoms that range from mild shortness of breath to acute respiratory failure. Approximately 56% of intensive care unit patients suffer from acute respiratory failure (ARF), with one-third of those subsequently dying.1 The pathogenesis of ARF can be classified to cardiogenic and non-cardiogenic pulmonary edema. Acute heart failure is the most common cause of increased hydrostatic pressure and is very prevalent, with almost 658,000 emergency department visits in the United States per year. The mortality rate from acute cardiogenic pulmonary edema ranges from 12% to 15%.2 In the USA, overall costs of heart failure in 2010 have been estimated at $39.2 billion, with hospitalization representing approximately 80% of direct treatment costs for heart failure.3
Acute lung injury (ALI) that is due to increased permeability pulmonary edema is also common, with an incidence of 86 per 100,000 person-years, and equates to over 190,000 cases and 74,500 fatalities annually in the United States. Although mortality has declined, recent studies still report an approximate 25% death rate.2
For many years, it was believed that fluid accumulation in the lung depends only on the abrogation of balanced Starling forces—the hydrostatic pressure and oncotic pressures.4,5 However, more recently it has been demonstrated that the alveolar epithelium has an active role in clearing edema out of the alveoli, a process called alveolar fluid clearance (AFC). |
The lung is responsible for gas exchange: enriching the circulation with oxygen (O2) and extruding carbon dioxide (CO2). The structure of the lungs is designed to facilitate gas exchange by enabling the transit of gases through the respiratory airways, and, as the gases reach alveolar sacs and alveolus clusters, gas exchange actually occurs. The alveoli are tightly wrapped with blood vessels allowing the diffusion of oxygen from the alveoli to the blood-stream of the alveolar blood vessels. Then, oxygenated blood is perfused throughout the body where gas exchange occurs in the capillary beds.6
Since gas exchange relies on diffusion, it is crucial that the layer separating the alveolar space from the interstitium is thin and permeable.6 To ensure this environment, alveoli are built of a monolayer epithelium that contains two alveolar epithelial cells (Figure 1), types I and II (AECI and AECII, respectively), and macrophages.7 Moreover, the alveolar space must be free of fluids and open. In utero, the fetal lung is filled with fluid that is removed shortly after birth, mainly because active reabsorption of sodium ions (Na+) across the alveolar epithelium creates an osmotic force favoring reabsorption of alveolar fluid.8 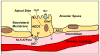 | Figure 1 Schematic Representation of Alveolar Epithelial Cells with the Components that Contribute to the Alveolar Fluid Clearance Process |
Alveolar epithelial cells type I are squamous with a diameter of about 50–100 μm; however, they are very thin, thus minimizing the diffusion distance between the alveolar airspace and the pulmonary capillaries, which facilitates gas exchange. Although they constitute 5%–10% of all lung cells,9 AECI cover more than 90% of the alveolar surface as they are very large and have thin cytoplasmic extensions. Recently, it was reported that AECI play an active role in water permeability and the regulation of alveolar fluid homeostasis.10,11
The alveolar epithelial cells type II are smaller and cuboidal, with a diameter of 21 μm in rats and 50 μm in humans. They occupy only ~5% of the surface area, yet AECII constitute ~15% of all lung cells and 60% of alveolar epithelial cells. They produce, secrete, and recycle lung surfactant; they transport ions, participate in lung immune responses, and can also be converted to AECI to repair damaged epithelium or during fetal lung development. The AECII has a distinct morphology with characteristic lamellar bodies and a bipolar plasma membrane, consisting of an apical side that has short microvilli and a basolateral domain. These cells contain a wide range of transport proteins, including epithelial Na+ channels (ENaCs), Na,K-ATPase, Na-H exchanger (NHE), and aquaporin-3 (AQP3).12–16 |
Normand et al. demonstrated that fetal lamb lungs can absorb fluid from the airspaces at birth,17 and by using 131I tracer Walters and Olver calculated the rate of lung liquid secretion in fetal lambs.18 Matthay et al. reported that mature sheep lungs have the ability to clear edema.19 Since then, alveolar fluid clearance has been extensively investigated.4,20–25 |
ALVEOLAR ACTIVE SODIUM TRANSPORT MECHANISM
Alveolar fluid clearance (AFC) is an active process carried out mostly by the apical epithelial Na+ channels, and the basolateral Na,K-ATPase is involved in AFC.13,24
Briefly, Na+ enters the alveolar epithelial cells through the apical amiloride-sensitive Na+ channels (ENaC), is transported through alveolar epithelial cells, and by a process that consumes energy is pumped out of the cell by the Na,K-ATPase located in the basolateral membrane in exchange for potassium entry in a ratio of 3:2 Na+–K+ against their chemical gradient. This active vectorial Na+ flux produces a transepithelial osmotic gradient that causes water to move from the airspaces following the gradient.8,26–28
Alveolar fluid reabsorption can be modulated by pharmacologic agents, gene therapy, and other interventions. Catecholamines, growth factors, vasopressin, aldosterone, overexpression of Na,K-ATPase subunits and chronic heart failure model increase alveolar fluid reabsorption (Figure 2A).16,29–40 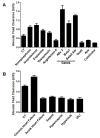 | Figure 2 The Effect of Various Pharmacologic and Pathophysiologic Conditions on Alveolar Fluid Clearance |
Active Na+ transport and edema clearance are inhibited by interventions that can be divided into several categories:
-
General AFC inhibitors such as the sodium channel blocker, amiloride,41 and the Na,K-ATPase inhibitor, ouabain.5
-
Consequences of acute lung injury (ALI), hypoxia,42 and hypercapnia that impair the alveolar epithelial function by increasing intracellular calcium levels.43,44
-
Mechanisms of ALI including sepsis,45 hyperoxia,46 high tidal volume ventilation and ventilation-induced lung injury,47 acute left atrial hypertension,48 andendothelin (Figure 2).49
Notably, aerosolized or intravenous β2-agonist therapy did not improve clinical outcomes in patients with lung injury; therefore, the use of β2-agonist therapy was not recommended in mechanically ventilated patients with lung injury.50,51 A more recent study appears to shed light onto the disparate effect of β2-agonist therapy in vitro in animals, and in patients with lung injury. Apparently, the β2-adrenergic receptor (β2AR) on alveolar macrophages can augment the release of IL-6, thus linking the sympathetic nervous system, by β2AR signaling, with lung inflammation and enhanced susceptibility to thrombotic cardiovascular events, which could have negative effects on the outcome of patients with acute lung injury.52
Alveolar fluid clearance reflects active sodium transport, and several mediators participate in this process, including Na,K-ATPase, Na+ channels, aquaporins, and others. The sodium–potassium pump, an energy-consuming enzyme (Na,K-ATPase), is a heterodimeric integral membrane protein that is synthesized in polysomes related to the rough endoplasmic reticulum. It is composed of two subunits: an α-subunit (a catalytic 110-kDa unit) and a β-subunit (a regulatory 55-kDa unit). The α-subunit contains binding sites for ATP hydrolysis, Na+, K+, and cardiac glycosides. There are at least three isoforms of α (α1, α2, and α3); they differ by their affinity to sodium, ouabain, and tissue distribution. The β-subunit is thought to be responsible for incorporating the α-subunit into the plasma membrane; there are three known isoforms of β-subunit.
A critical role is played by Na,K-ATPase in the homeostasis of Na+ and K+ during altered salt intake and pH regulation, besides its other important functions in several organs; therefore it is strictly regulated.8,53 The Na,K-ATPase can be regulated at the level of expression, internalization, and recruitment of the pump proteins to the basolateral membrane (Figure 3). For example, catecholamines enhance the ability of the lungs to clear edema by recruiting Na,K-ATPase from the alveolar epithelial cell (AEC) cytosol to the basolateral membranes within minutes and thus increasing the activity of the pump. This process is mediated via the cAMP/PKA pathway.30,54,55 In contrast, the adverse effects on AFC of hypoxia, hypercapnia, sepsis, and endothelin are due to the AMPK/PKC pathway, in which Na,K-ATPase is phosphorylated, leading to its endocytosis.42,45,49,56 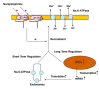 | Figure 3 Schematic Representation of Active Sodium Transport in the Alveolar Epithelial Cell Depicting Apical Na+ Channels, Basolaterally Located Na,K-ATPase, Aquaporins, and Co-transporters |
An important contributor to alveolar sodium transport is the sodium channel (ENaC). The ENaC is a heterotrimeric protein that can be composed by different combinations of three known subunits: αENaC, βENaC, and γENaC. Alveolar epithelial cells contain three types of channels with different selectivity properties: (1) a Ca2+-activated non-selective Na+ channel (NSC) composed of α-subunits alone, (2) a Na+-selective (moderately selective) channel composed of a combination of αENaC and βENaC or γENaC, and (3) the highly NSC composed of the three different subunits. The ENaC is located on the apical portion of AECs and plays a crucial role in sodium transport and AFC.28,30,57 Moreover, it has been shown that knocking out αENaC leads to defective AFC and premature death in newborn mice.
Water channels or aquaporins (AQPs) are expressed in the lungs. AQP1 is located in both the apical and basolateral aspects of endothelial cells and fibroblasts, and AQP3, AQP4, and AQP5 are expressed in the respiratory epithelium. In the human respiratory tract, AQP5 is expressed in the apical surface of AECI and AQP3 in the apical and basal membrane of AECII. Targeted deletions of AQPs in transgenic mice suggest that AQPs are not essential for alveolar fluid clearance; however, other compensatory mechanisms could have taken place instead of the deletion of AQPs.58
While the contribution of AECI to alveolar fluid clearance has been suggested, further studies to evaluate the role of distal epithelial cells are necessary. We also need to explore the role of chloride channels and their regulation, particularly in the pathways of activation as well as functional contributions of AQPs and cystic fibrosis transmembrane conductance regulator (CFTR). Additionally, signal transduction pathways as well as translation and post-translational pathways need to be studied as they may inform the field and help with novel therapies to modulate these pathways to enhance pulmonary edema clearance in patients with lung injury. |
The mechanisms of lung edema clearance contrast with the regulation of pulmonary edema formation. Clearance of edema fluid is an active process that requires active transport of Na+ out of alveolar airspaces, with water following the osmotic gradient. The regulation of vectorial Na+ transport across the alveolo-capillary barrier is mediated mostly by apical Na+ channels and basolaterally expressed Na,K-ATPases. In patients with acute respiratory distress syndrome and lung injury the mechanisms regulating alveolar fluid reabsorption are impaired, and the restoration of the alveolar epithelial function to keep the lungs dry is important for normal gas exchange to occur and patients to survive. More knowledge about the mechanisms regulating lung edema clearance is needed in order to develop novel therapeutic strategies to accelerate fluid clearance and improve the alveolar epithelial function. |
AEC |
alveolar epithelial cell |
AFC |
alveolar fluid clearance |
AQP |
aquaporin |
β2AR |
β2-adrenergic receptor |
CFTR |
cystic fibrosis transmembrane conductance regulator |
ENaC |
epithelial Na+ channel |
NHE |
Na-H exchanger |
NSC |
non-selective Na+ channel. |
|
|
3. Heidenreich PA, Albert NM, Allen LA, et al. Forecasting the impact of heart failure in the United States: a policy statement from the American Heart Association. Circ Heart Fail. 2013;6:606–19. http://dx.doi.org/10.1161/HHF.0b013e318291329a. 4. Berthiaume Y, Broaddus VC, Gropper MA, et al. Alveolar liquid and protein clearance from normal dog lungs. J Appl Physiol. 1988;65:585–93. 10. Dobbs LG, Johnson MD, Vanderbilt J, et al. The great big alveolar TI cell: evolving concepts and paradigms. Cell Physiol Biochem. 2010;25:55–62. http://dx.doi.org/10.1159/000272063. 11. Gonzalez R, Yang YH, Griffin C, et al. Freshly isolated rat alveolar type I cells, type II cells, and cultured type II cells have distinct molecular phenotypes. Am J Physiol Lung Cell Mol Physiol. 2005;288:L179–89. http://dx.doi.org/10.1152/ajplung.00272.2004. 12. Azzam ZS, Sznajder JI. The cellular mechanisms contributing to lung edema clearance. Isr Med Assoc J. 2000;2:235–9. 13. Matalon S, Benos DJ, Jackson RM. Biophysical and molecular properties of amiloride-inhibitable Na+ channels in alveolar epithelial cells. Am J Physiol. 1996;271:L1–22. 19. Matthay MA, Landolt CC, Staub NC. Differential liquid and protein clearance from the alveoli of anesthetized sheep. J Appl Physiol. 1982;53:96–104. 20. Serikov VB, Grady M, Matthay MA. Effect of temperature on alveolar liquid and protein clearance in an in situ perfused goat lung. J Appl Physiol. 1993;75:940–7. 21. Smedira N, Gates L, Hastings R, et al. Alveolar and lung liquid clearance in anesthetized rabbits. J Appl Physiol. 1991;70:1827–35. 22. Ballard ST, Schepens SM, Falcone JC, et al. Regional bioelectric properties of porcine airway epithelium. J Appl Physiol. 1992;73:2021–7. 23. Effros RM, Mason GR, Sietsema K, et al. Pulmonary epithelial sieving of small solutes in rat lungs. J Appl Physiol. 1988;65:640–8. 24. Rutschman DH, Olivera W, Sznajder JI. Active transport and passive liquid movement in isolated perfused rat lungs. J Appl Physiol. 1993;75:1574–80. 32. Barquin N, Ciccolella DE, Ridge KM, et al. Dexamethasone upregulates the Na-K-ATPase in rat alveolar epithelial cells. Am J Physiol. 1997;273:L825–30. 33. Berthiaume Y, Staub NC, Matthay MA. Beta-adrenergic agonists increase lung liquid clearance in anesthetized sheep. J Clin Invest. 1987;79:335–43. http://dx.doi.org/10.1172/JCI112817. 34. Dumasius V, Sznajder JI, Azzam ZS, et al. beta(2)-Adrenergic receptor overexpression increases alveolar fluid clearance and responsiveness to endogenous catecholamines in rats. Circ Res. 2001;89:907–14. http://dx.doi.org/10.1161/hh2201.100204. 36. Saldias F, Lecuona E, Friedman E, et al. Modulation of lung liquid clearance by isoproterenol in rat lungs. Am J Physiol. 1998;274:L694–701. 38. Olivera W, Ridge K, Wood LD, et al. Active sodium transport and alveolar epithelial Na-K-ATPase increase during subacute hyperoxia in rats. Am J Physiol. 1994;266:L577–84. 39. Guetta J, Klorin G, Tal R, et al. Vasopressin-2 receptor antagonist attenuates the ability of the lungs to clear edema in an experimental model. Am J Respir Cell Mol Biol. 2012;47:583–8. http://dx.doi.org/10.1165/rcmb.2012-0117OC. 40. Azzam ZS, Dumasius V, Saldias FJ, et al. Na,K-ATPase overexpression improves alveolar fluid clearance in a rat model of elevated left atrial pressure. Circulation. 2002;105:497–501. http://dx.doi.org/10.1161/hc0402.102848. 42. Dada LA, Chandel NS, Ridge KM, et al. Hypoxia-induced endocytosis of Na,K-ATPase in alveolar epithelial cells is mediated by mitochondrial reactive oxygen species and PKC-zeta. J Clin Invest. 2003;111:1057–64. http://dx.doi.org/10.1172/JCI16826. 43. Briva A, Vadasz I, Lecuona E, et al. High CO2 levels impair alveolar epithelial function independently of pH. PLoS One. 2007;2:e1238. 44. Briva A, Santos C, Malacrida L, et al. Adenosine triphosphate-dependent calcium signaling during ventilator-induced lung injury is amplified by hypercapnia. Exp Lung Res. 2011;37:471–81. http://dx.doi.org/10.3109/01902148.2011.598217. 48. Saldias FJ, Azzam ZS, Ridge KM, et al. Alveolar fluid reabsorption is impaired by increased left atrial pressures in rats. Am J Physiol Lung Cell Mol Physiol. 2001;281:L591–7. 50. Gao Smith F, Perkins GD, Gates S, et al. Effect of intravenous beta-2 agonist treatment on clinical outcomes in acute respiratory distress syndrome (BALTI-2): a multicentre, randomised controlled trial. Lancet. 2012;379:229–35. http://dx.doi.org/10.1016/S0140-6736(11)61623-1. 51. Matthay MA, Brower RG, Carson S, et al. Randomized, placebo-controlled clinical trial of an aerosolized beta(2)-agonist for treatment of acute lung injury. Am J Respir Crit Care Med. 2011;184:561–8. http://dx.doi.org/10.1164/rccm.201012-2090OC. 52. Chiarella SE, Soberanes S, Urich D, et al. beta(2)-Adrenergic agonists augment air pollution-induced IL-6 release and thrombosis. J Clin Invest. 2014;124:2935–46. http://dx.doi.org/10.1172/JCI75157. 54. Bertorello AM, Ridge KM, Chibalin AV, et al. Isoproterenol increases Na+-K+-ATPase activity by membrane insertion of alpha-subunits in lung alveolar cells. Am J Physiol. 1999;276:L20–7. 55. Ridge KM, Dada L, Lecuona E, et al. Dopamine-induced exocytosis of Na,K-ATPase is dependent on activation of protein kinase C-epsilon and −delta. Mol Biol Cell. 2002;13:1381–9. http://dx.doi.org/10.1091/mbc.01-07-0323. 56. Gusarova GA, Dada LA, Kelly AM, et al. Alpha1-AMP-activated protein kinase regulates hypoxia-induced Na,K-ATPase endocytosis via direct phosphorylation of protein kinase C zeta. Mol Cell Biol. 2009;29:3455–64. http://dx.doi.org/10.1128/MCB.00054-09. 57. Norlin A, Finley N, Abedinpour P, et al. Alveolar liquid clearance in the anesthetized ventilated guinea pig. Am J Physiol. 1998;274:L235–43. 58. Dobbs LG, Gonzalez R, Matthay MA, et al. Highly water-permeable type I alveolar epithelial cells confer high water permeability between the airspace and vasculature in rat lung. Proc Natl Acad Sci U S A. 1998;95:2991–6. http://dx.doi.org/10.1073/pnas.95.6.2991. |