TRANSCATHETER AORTIC VALVE IMPLANTATION
Transcatheter aortic valve implantation is an alternative to surgical aortic valve replacement (SAVR). A stented bioprosthetic valve is delivered via catheterization and deployed inside the stenotic native valve.25,26 In the latest generation of devices, the only US Food and Drug Administration (FDA)-approved TAVI devices are the balloon-expandable Sapien 3 Ultra (Edwards Lifesciences Corp., Irvine, CA, USA), the self-expandable Evolut Pro (Medtronic, Minneapolis, MN, USA), and the self-expandable and mechanically locked Lotus Edge (Boston Scientific, Marlborough, MA, USA).27 In addition to these three devices, numerous other TAVI devices (including the Portico from Abbott, the Acurate Neo from Boston Scientific, and JenaValve’s device) have received Conformité Européenne (CE) marks16; however, most of them have been discontinued. While each of the various CE-marked devices has its own advantages, these advantages are usually related to aspects other than valve hemodynamics. Although TAVI was originally approved for only high-risk surgical patients, it is now approved for low-risk surgical patients.28 This recent change could increase the annual number of TAVI procedures from 180,000 to 270,000 in Europe and North America.29 In addition to the classical use of TAVI in CAVD, both self-expanding and balloon-expandable TAVI devices are FDA-approved for ViV implantation.30 However, the suture ring of the SAVR makes it narrower than the native root, and inserting a TAVI reduces the orifice area of the valve even more, thereby harming hemodynamic performance.31 Additionally, some of the TAVI complications are more common in ViV (discussed in the next section). Transcatheter aortic valve implantation is also being used in bileaflet aortic valve patients at high surgical risk, although such use has not yet been approved for this population.32 The risk of various hemodynamic complications is also higher than in CAVD patients, due to the non-circular anatomical shape of the aortic valve in these patients.
Hemodynamic Complications The new-generation TAVI devices demonstrates a vast decrease in complications; however, the existence of some adverse outcomes remains a concern due to the shift toward use in lower-risk patients. 31 Some of these complications include conduction abnormalities (necessitating permanent pacemaker implantation), coronary artery obstruction (CAO), paravalvular leak (PVL), and valve thrombosis. 31 The two last-mentioned are direct hemodynamic complications.
Paravalvular leak. Paravalvular leak is a leakage through the gaps between the implanted stent of TAVI devices and the native valve (Figure 2). This adverse hemodynamic outcome has been significantly minimized in the latest-generation devices, from a prevalence in patients of 25% to 5%.16 This reduction was achieved by adding an outer skirt or cuff that covers the ventricular portion of the stent. In the original Sapien 3 valve, the outer skirt included openings that created pockets that could fill with blood, thereby sealing the paravalvular gaps.16 This design was later refined in the Sapien 3 Ultra valve by increasing the outer skirt height, closing the pockets, and adding texture to the polyethylene terephthalate fabric. While the latest self-expandable devices also have an outer skirt, they can seal the gaps with an optimized anatomical fitting, specifically by having a larger stent diameter on the ventricular side than in the valve region. In addition to design improvements in the latest devices, if aortic regurgitation (AR) is found immediately following implantation, PVL is minimized by post-dilation with ballooning. These advances significantly reduced the incidence of AR post-TAVI; however, moderate-to-severe AR is still much more frequent compared to SAVR.35 Since PVL is a very patient-specific complication, from an engineering point of view, it is more useful to evaluate it with computational fluid dynamics (CFD) than with bench experiments.36 In CFD, the basic equations that describe the flow of a fluid are solved by computational software. Therefore, CFD enables virtual replication of procedural options that cannot be tested in vitro for specific patients. Several studies have used CFD37–41 and fluid-structure interaction,42 where the fluid dynamics equations are coupled with solid mechanics models, to estimate PVL. This is also one of the features of a commercial service for pre-procedural planning based on patient-specific scans, as described below (Patient-specific Pre-procedural Planning Based on Numerical Models). 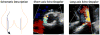 | Figure 2 Paravalvular Leak Post-Transcatheter Aortic Valve Implantation (TAVI) |
Thrombogenicity. Hemodynamics is one of the main contributing factors for thrombosis.43,44 Exposure of platelets to elevated flow stresses and platelet adhesion in stagnant flow regions are considered the two main mechanical causes of thrombogenicity.45,46 In heart valves, these two factors, along with non-hemodynamic factors like hemocompatibility, can cause leaflet thrombosis or thromboemboli. In mechanical valves, the main concern is thrombus formation resulting from disturbed flow and elevated shear stress in the regurgitant flow through the narrow gaps. While it is true that the narrow gaps in PVL around TAVI can also cause this type of thrombus formation,40,47 obviously the leakage itself is usually a bigger concern than the thrombosis and constitutes the rationale behind performing post-TAVI dilation. On the other hand, blood flow stagnation in the valvular region is the suggested cause of leaflet thrombosis in TAVI, both in clinical studies48 and based on in vitro particle image velocimetry (PIV) measurements of the flow velocity vector field (Figure 3).50,51 Specifically, leaflet thrombosis is the assumed reason for reduced leaflet motion,52 as a result of hypoattenuated leaflet thickening.53 The prevalence of leaflet thrombosis remains unknown since cases that have not been diagnosed clinically have been discovered in pathological valve studies,48 but frequencies of 16% in Sapien 3, 8% in Evolut, and 14% in Lotus TAVI devices as compared with 4% in SAVR patients have been suggested.52 Additionally, the occurrence of leaflet thrombosis post-ViV placement was reported to be six times the occurrence of leaflet thrombosis post-TAVI in native valve.54 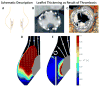 | Figure 3 Flow Stasis Post-Transcatheter Aortic Valve Implantation (TAVI) and Leaflet Thickening That Indicates Thrombosis Location |
Several attempts have been made to study the hemodynamic causes of hypoattenuated leaflet thickening by engineering methods, both experimental and numerical. Experimental studies compared the native valve to TAVI,50 surgical valve to ViV51 with idealized geometry, or surgical valve to ViV with commercial valves.55 Numerical studies that compared surgical valve to ViV56–58 by CFD with prescribed leaflets motion also employed idealized geometry that were experimentally measured.57,58 All these studies demonstrated that valve confinement, where the TAVI valve is surrounded by the previous leaflets of the native or the degenerated SAVR valve, can increase the blood residence time near the leaflets. Therefore, supra-annular implantation, like in Evolut (where the TAVI valve is only partially confined), is expected to have a lower thrombogenic risk than a fully confined valve, such as the intra-annularly implanted Sapien.58 A recently proposed method to address CAO is to lacerate the leaflets of the bio-prosthetic valve by a technique known as BASILICA (bioprosthetic or native aortic scallop intentional laceration to prevent coronary artery obstruction)59 (see below, Hemodynamics of structural complications). In addition to the original intention of this technique, engineering studies suggest that the laceration can allow better washout and reduce the flow stagnation in the valvular region, thus leading to lower thrombogenic risk.60,61
Hemodynamics of structural complications. In addition to these two direct hemodynamic complications, the other TAVI complications also affect blood flow. Coronary artery obstruction obviously has a major effect on coronary hemodynamics. It is more common in ViV cases than in classical TAVI, and is related the to the surgical valve design, with a complication rate of up to 5.3% for externally mounted surgical valves.62,63 Patients suspected of being at risk for CAO, based on pre-procedural imaging, should be protected pre-emptively by “chimney” stenting.64 An alternative to chimney stenting is the BASILICA technique59 where the laceration directly prevents obstruction. While CAO risk is currently evaluated based only on the anatomic location of the coronary ostia, numerical models can help to quantitatively assess flow dynamics.65,66 The circularity and size of the valve orifice can be highly dependent on patient anatomy, especially with self-expandable TAVI devices. Clearly, it is undesirable to have a non-circular valve, and this phenomenon has been generally been addressed by the supra-annular design of the TAVI device.16 Finally, valve embolization (migration) exemplifies structural complications due to hemodynamics. While it is relatively rare (occurrence as low as 0.5%)67 and considered a consequence of insufficient anchoring, valve embolization is a direct result of the diastolic blood pressure pushing the valve into the ventricle. Recently, we suggested that BASILICA can weaken anchorage forces, although our study did not indicate that it was weakened enough to cause migration.68 Stronger anchoring forces, for example by over-inflating the balloon-expandable device, can obviously help prevent migration. Nevertheless, these approaches can cause additional non-hemodynamic complications such as conduction abnormalities (necessitating permanent pacemaker implantation) or even aortic root rupture.
Patient-specific Pre-procedural Planning Based on Numerical Models In recent years, several numerical models have been approved for patient-specific procedural planning in various medical treatments. 69 The FEops HEARTguide (FEops nv, Gent, Belgium) is a CE-marked service for making pre-interventional TAVI device size and position recommendations based on patient-specific numerical models. The recommendations are based on both finite element analysis of the implantation 70 and CFD analysis of the post-procedural PVL. 37 To utilize this service, clinicians send routine computed tomography (CT) scans of the patient to the company. The company then reconstructs the cardiac anatomy, generates and runs the finite element analysis and CFD simulations, and provides a report on the results of several scenarios within two working days. Since the first presentation of this tool, numerous studies have demonstrated its clinical usage including for implantation in bicuspid aortic valves, 71–73 and the use of TAVI in the mitral location with native calcified valves 74,75 and inside a failed bioprosthetic valve. 76 Use of the FEops HEARTguide has also been expanded to additional TAVI devices, 77 device optimization, 78 and also for procedural recommendations based on additional possible complications, such as conduction abnormalities. 79 From a hemodynamic perspective, the most important capability of this tool is obviously calculation of PVL ( Figure 4), which demonstrated good predictions in a clinical study of 60 patients. 37 On the other hand, in a study that used the FEops HEARTguide to compare coronary flow with several TAVI orientations, 38 there was no difference between the flow results in the various cases. 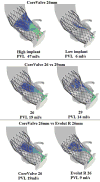 | Figure 4 Patient-specific Pre-procedural Paravalvular Leak Calculations |
|
Mitral valve regurgitation (MVR) is a leakage due to improper closure of the valve. It is the most common valvular heart disease with a prevalence of approximately 1.7% in the adult population.81 Due to its increased prevalence with age and the growing aging population, the number of cases in the year 2030 is expected to be almost double that of the year 2000.82 Mitral valve regurgitation is caused by either a valve prolapse (primary MVR, due to a degenerative abnormality of the leaflets, chordae tendineae, papillary muscles, or the mitral annulus) or a left ventricular dysfunction (secondary or functional MVR).83 Current treatments include mostly surgical valve repair or replacement.84 Valve replacement involves replacing the native valve with a prosthetic one. Obviously, implantation orientation significantly influences the flow pattern, especially with mechanical valves, but it is less clear what the optimal orientation is.85 In a valve repair, the native valve remains in place, and the leaflets, chordae, and papillary muscle are manipulated to restore normal valve behavior with a stabilized annulus while preserving the valve’s orifice size and left ventricular function.86,87 Repair techniques are based on annuloplasty, resection, addition of artificial chordae, or a combination thereof.88,89 Annuloplasty, with either rigid or flexible rings or bands, is necessary in most repairs.90 Valve repair is recommended for patients with primary MVR90 since the repair has a low mortality rate91 and it improves ventricular function with no need for anticoagulation. However, there remains a risk of residual MVR and concerns regarding mitral valve repair durability.92
Unfortunately, the majority of severe MVR patients are not treated due to the high surgical risk, leading to considerable morbidity and mortality.93,94 As a consequence, these patients can only be treated percutaneously. There is one such device that has been approved for MVR by the FDA, the MitraClip (Abbott Laboratories, Abbott Park, Illinois, USA). This transcatheter procedure involves the implantation of a clip that grasps both the anterior and the posterior leaflets of the mitral valve, mimicking surgical edge-to-edge valve repair that is done via open heart surgery.95 However, the procedural results are often suboptimal even in patients who meet the inclusion criteria, i.e. severely symptomatic secondary MVR patients.96 The Pascal system (Edwards Lifesciences Corporation, Irvine, CA), which is still in clinical trials, is another edge-to-edge valve repair device that aims to tackle some of these limitations by including wider paddles and a central spacer.97 Other percutaneous CE-marked interventions are based on direct (Mitralign System, from Mitralign, Inc., Tewksbury, MA, USA; Cardioband, from Edwards Lifesciences) and indirect (Carillon System, from Cardiac Dimensions, Inc., Kirkland, WA, USA) mitral annuloplasty.95,98
Transcatheter mitral valve replacement (TMVR) is a potential alternative to surgical treatment for a wide range of pathologies that cannot currently be treated percutaneously.95 However, TMVR has unique challenges, such as the size and shape of the valve, lack of calcification deposits for anchorage, high hemodynamic pressures, and complex sub-valvular apparatus. These challenges led to a limited clinical experience with such devices.93 A significant effort is being made to develop TMVR devices, with more than 10 currently at various stages of development.99–101 These TMVR devices have several mechanisms for anchorage and sufficient sealing around the device. Unlike TAVI, the main anchoring challenge focuses on not applying strong radial forces, which can obstruct and damage the aortic valve.101 Suggested TMVR anchoring mechanisms include counteracting axial forces by using ventricular tethers, native valve anchors, atrial and ventricular flanges, sub-annular hooks, atrial cages, and implantation of a docking system. From a hemodynamic perspective, all these anchoring mechanisms contribute to some flow disturbances. Nevertheless, flow through the valve itself should be similar to the flow through any other type of bioprosthetic valve. Another possible procedural complication with a direct hemodynamic effect is left ventricular outflow tract obstruction. For these cases, laceration of the anterior mitral leaflet to prevent outflow obstruction102,103 was suggested, a technique similar to using BASILICA in a TAVI.
Several studies used engineering methods to experimentally study the hemodynamics of the mitral valve, including MVR before and after treatment.104–106 Numerical methods have also been employed to model healthy and diseased mitral valves, before and after surgery.107–112 Both mitral annuloplasty113–118 and edge-to-edge procedures119–121 have been modeled with finite element analysis to evaluate their effect on tissue stress, tension in the chordae tendineae, and hemodynamics. Most numerical models of percutaneous MVR treatments focused on evaluating the commercially available MitraClip.105,119–122 Sturla et al.105 studied the effect of MitraClip implantation with both in vitro experiments and numerical models. While hemodynamics was not modeled numerically, it was measured experimentally. Therefore, this study was able to find a correlation between the “dry” experimental results and hemodynamics. Kamakoti et al.122 presented numerical simulations of fluid structure interaction in the mitral valve post-MitraClip implantation (Figure 5). Their main focus was regurgitation reduction using the MitraClip, and their results indicated the importance of the grasping location. While no study presented numerical simulations of TMVR devices, both Karady et al.74 and Serban et al.75 described use of the FEops HEARTguide to model TAVI devices (the Lotus valve from Boston Scientific, and the Sapien 3 from Edwards Lifesciences, respectively) in the mitral location. In these cases, patients suffered from severe MVR and mitral valve stenosis with significant mitral valve annulus calcification. This specific pathology enabled the use of TAVI devices rather than a dedicated TMVR device. 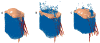 | Figure 5 Comparison of Calculated Leakage in the Mitral Valve |
|
1. Beyar R. High technology in medicine: lessons from cardiovascular innovations and future perspective. Rambam Maimonides Med J. 2013;4:e0009. https://doi.org/10.5041/RMMJ.10109. 3. De Paulis R, De Matteis GM, Nardi P, Scaffa R, Buratta MM, Chiariello L. Opening and closing characteristics of the aortic valve after valve-sparing procedures using a new aortic root conduit. Ann Thorac Surg. 2001;72:487–94. https://doi.org/10.1016/s0003-4975(01)02747-3. 5. Halevi R, Hamdan A, Marom G, et al. Fluid-structure interaction modeling of calcific aortic valve disease using patient-specific three-dimensional calcification scans. Med Biol Eng Comput. 2016;54:1683–94. https://doi.org/10.1007/s11517-016-1458-0. 6. Spühler JH, Jansson J, Jansson N, Hoffman J. 3D fluid-structure interaction simulation of aortic valves using a unified continuum ALE FEM model. Front Physiol. 2018;9:363. https://doi.org/10.3389/fphys.2018.00363. 7. Capelli C, Corsini C, Biscarini D, et al. Pledget-armed sutures affect the haemodynamic performance of biologic aortic valve substitutes: a preliminary experimental and computational study. Cardiovasc Eng Technol. 2017;8:17–29. https://doi.org/10.1007/s13239-016-0284-8. 8. Ghanbari H, de Mel A, Seifalian AM. Cardiovascular application of polyhedral oligomeric silsesquioxane nanomaterials: a glimpse into prospective horizons. Int J Nanomedicine. 2011;6:775–86. https://doi.org/10.2147/IJN.S14881. 9. Sanders B, Loerakker S, Fioretta ES, et al. Improved geometry of decellularized tissue engineered heart valves to prevent leaflet retraction. Ann Biomed Eng. 2016;44:1061–71. https://doi.org/10.1007/s10439-015-1386-4. 10. Marom G, Chiu WC, Crosby JR, et al. Numerical model of full-cardiac cycle hemodynamics in a total artificial heart and the effect of its size on platelet activation. J Cardiovasc Transl Res. 2014;7:788–96. https://doi.org/10.1007/s12265-014-9596-y. 12. Kytö V, Myllykangas ME, Sipilä J, Niiranen TJ, Rautava P, Gunn J. Long-term outcomes of mechanical vs biologic aortic valve prosthesis in patients older than 70 years. Ann Thorac Surg. 2019;108:1354–60. https://doi.org/10.1016/j.athoracsur.2019.04.012. 13. Witkowski A, Jastrzebski J, Dabrowski M, Chmielak Z. Second transcatheter aortic valve implantation for treatment of suboptimal function of previously implanted prosthesis: review of the literature. J Interv Cardiol. 2014;27:300–7. https://doi.org/10.1111/joic.12120. 14. Saji M, Tobaru T, Higuchi R, Takanashi S, Takayama M, Isobe M. Repeat transcatheter aortic valve replacement using a 23 mm Evolut R in a small patient with a failed 20 mm SAPIEN XT. Cardiovasc Interv Ther. 2019;34:80–2. https://doi.org/10.1007/s12928-018-0519-8. 20. Rotman OM, Kovarovic B, Chiu WC, et al. Novel polymeric valve for transcatheter aortic valve replacement applications: in vitro hemodynamic study. Ann Biomed Eng. 2019;47:113–25. https://doi.org/10.1007/s10439-018-02119-7. 21. Ghosh RP, Marom G, Rotman OM, et al. Comparative fluid-structure interaction analysis of polymeric transcatheter and surgical aortic valves’ hemodynamics and structural mechanics. J Biomech Eng. 2018 Jun 25; [Epub ahead of print]. https://doi.org/10.1115/1.4040600. 24. Zhang BL, Bianco RW, Schoen FJ. Preclinical assessment of cardiac valve substitutes: current status and considerations for engineered tissue heart valves. Front Cardiovasc Med. 2019;6:72. https://doi.org/10.3389/fcvm.2019.00072. 25. Cao C, Ang SC, Indraratna P, et al. Systematic review and meta-analysis of transcatheter aortic valve implantation versus surgical aortic valve replacement for severe aortic stenosis. Ann Cardiothorac Surg. 2013;2:10–23. https://doi.org/10.3978/j.issn.2225-319X.2012.11.09. 28. Wu C, Vasseur B, Maisel W. The march of transcatheter aortic valve replacement therapy—US Food and Drug Administration perspectives on device approval for patients at low surgical risk. JAMA Cardiol. 2020;5:5–6. https://doi.org/10.1001/jamacardio.2019.4383. 29. Durko AP, Osnabrugge RL, Van Mieghem NM, et al. Annual number of candidates for transcatheter aortic valve implantation per country: current estimates and future projections. Eur Heart J. 2018;39:2635–42. https://doi.org/10.1093/eurheartj/ehy107. 30. Saxon JT, Allen KB, Cohen DJ, Chhatriwalla AK. Bioprosthetic valve fracture during valve-in-valve TAVR: bench to bedside. Interv Cardiol. 2018;13:20–6. https://doi.org/10.15420/icr.2017:29:1. 32. Makkar RR, Yoon SH, Leon MB, et al. Association between transcatheter aortic valve replacement for bicuspid vs tricuspid aortic stenosis and mortality or stroke. JAMA. 2019;321:2193–202. https://doi.org/10.1001/jama.2019.7108. 33. Di Martino LF, Vletter WB, Ren B, et al. Prediction of paravalvular leakage after transcatheter aortic valve implantation. Int J Cardiovasc Imaging. 2015;31:1461–8. https://doi.org/10.1007/s10554-015-0703-1. 37. de Jaegere P, De Santis G, Rodriguez-Olivares R, et al. Patient-specific computer modeling to predict aortic regurgitation after transcatheter aortic valve replacement. JACC Cardiovasc Interv. 2016;9:508–12. https://doi.org/10.1016/j.jcin.2016.01.003. 38. Fuchs A, Kofoed KF, Yoon SH, et al. Commissural alignment of bioprosthetic aortic valve and native aortic valve following surgical and transcatheter aortic valve replacement and its impact on valvular function and coronary filling. JACC Cardiovasc Interv. 2018;11:1733–43. https://doi.org/10.1016/j.jcin.2018.05.043. 39. Mao W, Wang Q, Kodali S, Sun W. Numerical parametric study of paravalvular leak following a transcatheter aortic valve deployment into a patient-specific aortic root. J Biomech Eng. 2018;140:101007–11. https://doi.org/10.1115/1.4040457. 40. Bianchi M, Marom G, Ghosh RP, et al. Patient-specific simulation of transcatheter aortic valve replacement: impact of deployment options on paravalvular leakage. Biomech Model Mechanobiol. 2019;18:435–51. https://doi.org/10.1007/s10237-018-1094-8. 41. Lavon K, Marom G, Bianchi M, et al. Biomechanical modeling of transcatheter aortic valve replacement in a stenotic bicuspid aortic valve: deployments and paravalvular leakage. Med Biol Eng Comput. 2019;57:2129–43. https://doi.org/10.1007/s11517-019-02012-y. 42. Luraghi G, Migliavacca F, Garcia-Gonzalez A, et al. On the modeling of patient-specific transcatheter aortic valve replacement: a fluid-structure interaction approach. Cardiovasc Eng Technol. 2019;10:437–55. https://doi.org/10.1007/s13239-019-00427-0. 44. Kumar DR, Hanlin E, Glurich I, Mazza JJ, Yale SH. Virchow’s contribution to the understanding of thrombosis and cellular biology. Clin Med Res. 2010;8:168–72. https://doi.org/10.3121/cmr.2009.866. 46. Marom G, Bluestein D. Lagrangian methods for blood damage estimation in cardiovascular devices--How numerical implementation affects the results. Expert Rev Med Devices. 2016;13:113–22. https://doi.org/10.1586/17434440.2016.1133283. 49. Rosseel L, De Backer O, Sondergaard L. Clinical valve thrombosis and subclinical leaflet thrombosis following transcatheter aortic valve replacement: is there a need for a patient-tailored antithrombotic therapy? Front Cardiovasc Med. 2019;6:44. https://doi.org/10.3389/fcvm.2019.00044. 50. Ducci A, Pirisi F, Tzamtzis S, Burriesci G. Transcatheter aortic valves produce unphysiological flows which may contribute to thromboembolic events: an in-vitro study. J Biomech. 2016;49:4080–9. https://doi.org/10.1016/j.jbiomech.2016.10.050. 52. Chakravarty T, Sondergaard L, Friedman J, et al. Subclinical leaflet thrombosis in surgical and transcatheter bioprosthetic aortic valves: an observational study. Lancet. 2017;389:2383–92. https://doi.org/10.1016/S0140-6736(17)30757-2. 54. Jose J, Sulimov DS, El-Mawardy M, et al. Clinical bioprosthetic heart valve thrombosis after transcatheter aortic valve replacement: incidence, characteristics, and treatment outcomes. JACC Cardiovasc Interv. 2017;10:686–97. https://doi.org/10.1016/j.jcin.2017.01.045. 55. Hatoum H, Moore BL, Maureira P, Dollery J, Crestanello JA, Dasi LP. Aortic sinus flow stasis likely in valve-in-valve transcatheter aortic valve implantation. J Thorac Cardiovasc Surg. 2017;154:32–43.e1. https://doi.org/10.1016/j.jtcvs.2017.03.053. 57. Vahidkhah K, Barakat M, Abbasi M, et al. Valve thrombosis following transcatheter aortic valve replacement: significance of blood stasis on the leaflets. Eur J Cardiothorac Surg. 2017;51:927–35. https://doi.org/10.1093/ejcts/ezw407. 59. Khan JM, Dvir D, Greenbaum AB, et al. Transcatheter laceration of aortic leaflets to prevent coronary obstruction during transcatheter aortic valve replacement: concept to first-in-human. JACC Cardiovasc Interv. 2018;11:677–89. https://doi.org/10.1016/j.jcin.2018.01.247. 61. Khodaee F, Qiu D, Dvir D, Azadani AN. Reducing the risk of leaflet thrombosis in transcatheter aortic valve-in-valve implantation by BASILICA: a computational simulation study. EuroIntervention. 2019;15:67–70. https://doi.org/10.4244/EIJ-D-19-00048. 62. Mosquera VX, Gonzalez-Barbeito M, Bouzas-Mosquera A, et al. Efficacy and safety of transcatheter valve-in-valve replacement for Mitroflow bioprosthetic valve dysfunction. J Card Surg. 2018;33:356–62. https://doi.org/10.1111/jocs.13720. 63. Cheung AW, Ye J, Dvir D, Wood DA, Webb JG. Aortic valve-in-valve in externally mounted bioprosthesis: a safe treatment option for bioprosthetic structural valve dysfunction. Innovations (Phila). 2018;13:171–6. https://doi.org/10.1097/IMI.0000000000000500. 64. Spaziano M, Akodad M, Hovasse T, Lefevre T, Bouvier E, Chevalier B. Simultaneous TAVR and left main “chimney” stenting in a patient with low left main height. JACC Cardiovasc Interv. 2017;10:e185–7. https://doi.org/10.1016/j.jcin.2017.06.047. 65. Heitkemper M, Hatoum H, Azimian A, et al. Modeling risk of coronary obstruction during transcatheter aortic valve replacement. J Thorac Cardiovasc Surg. 2020;159:829–38.e3. https://doi.org/10.1016/j.jtcvs.2019.04.091. 68. Yaakobovich H, Plitman Mayo R, Zaretsky U, Finkelstein A, Marom G. Numerical models of valve-in-valve implantation: effect of intentional leaflet laceration on the anchorage. Biomech Model Mechanobiol. 2020;19:415–26. https://doi.org/10.1007/s10237-019-01218-1. 70. Schultz C, Rodriguez-Olivares R, Bosmans J, et al. Patient-specific image-based computer simulation for the prediction of valve morphology and calcium displacement after TAVI with the Medtronic Corevalve and the Edwards Sapien valve. EuroIntervention. 2016;11:1044–52. https://doi.org/10.4244/EIJV11I9A212. 71. Brouwer J, Gheorghe L, Nijenhuis VJ, et al. Insight on patient specific computer modeling of transcatheter aortic valve implantation in patients with bicuspid aortic valve disease. Catheter Cardiovasc Interv. 2019;93:1097–105. https://doi.org/10.1002/ccd.27990. 72. Dowling C, Bavo AM, El Faquir N, et al. Patient-specific computer simulation of transcatheter aortic valve replacement in bicuspid aortic valve morphology. Circ Cardiovasc Imaging. 2019;12:e009178. https://doi.org/10.1161/CIRCIMAGING.119.009178. 73. Dowling C, Firoozi S, Brecker SJ. First-in-human experience with patient-specific computer simulation of TAVR in bicuspid aortic valve morphology. JACC Cardiovasc Interv. 2020;13:184–92. https://doi.org/10.1016/j.jcin.2019.07.032. 74. Karady J, Ntalas I, Prendergast B, et al. Transcatheter mitral valve replacement in mitral annulus calcification - “The art of computer simulation”. J Cardiovasc Comput Tomogr. 2018;12:153–7. https://doi.org/10.1016/j.jcct.2017.12.007. 75. Serban R, Redwood S, Prendergast B, Rajani R. Real-time image integration for transcatheter mitral valve replacement in mitral annular calcification. J Thorac Cardiovasc Surg. 2019;157:e135–9. https://doi.org/10.1016/j.jtcvs.2018.09.029. 76. Adams HSL, Rajani R, Hildick-Smith D, Redwood S. “Between a rock and the mitral valve space”: transcatheter mitral valve-in-valve implantation for paravalvular leak and refractory hemolysis complicated by circumflex coronary occlusion. Catheter Cardiovasc Interv. 2019 Nov 6; [Epub ahead of print]. https://doi.org/10.1002/ccd.28573. 77. Rocatello G, El Faquir N, de Backer O, et al. The impact of size and position of a mechanical expandable transcatheter aortic valve: novel insights through computational modelling and simulation. J Cardiovasc Transl Res. 2019;12:435–46. https://doi.org/10.1007/s12265-019-09877-2. 78. Rocatello G, De Santis G, De Bock S, De Beule M, Segers P, Mortier P. Optimization of a transcatheter heart valve frame using patient-specific computer simulation. Cardiovasc Eng Technol. 2019;10:456–68. https://doi.org/10.1007/s13239-019-00420-7. 79. Rocatello G, El Faquir N, De Santis G, et al. Patient-specific computer simulation to elucidate the role of contact pressure in the development of new conduction abnormalities after catheter-based implantation of a self-expanding aortic valve. Circ Cardiovasc Interv. 2018;11:e005344. https://doi.org/10.1161/CIRCINTERVENTIONS.117.005344. 80. El Faquir N, Ren B, Van Mieghem NM, Bosmans J, de Jaegere PP. Patient-specific computer modelling - its role in the planning of transcatheter aortic valve implantation. Neth Heart J. 2017;25:100–5. https://doi.org/10.1007/s12471-016-0923-6. 83. Asgar AW, Mack MJ, Stone GW. Secondary mitral regurgitation in heart failure: pathophysiology, prognosis, and therapeutic considerations. J Am Coll Cardiol. 2015;65:1231–48. https://doi.org/10.1016/j.jacc.2015.02.009. 84. Feldman T, Kar S, Rinaldi M, et al. Percutaneous mitral repair with the MitraClip system: safety and midterm durability in the initial EVEREST (endovascular valve edge-to-edge repair study) cohort. J Am Coll Cardiol. 2009;54:686–94. https://doi.org/10.1016/j.jacc.2009.03.077. 85. Saaid H, Segers P, Novara M, Claessens T, Verdonck P. Single calibration multiplane stereo-PIV: the effect of mitral valve orientation on three-dimensional flow in a left ventricle model. Exp Fluids. 2018;59:49. https://doi.org/10.1007/s00348-018-2504-5. 86. Lamelas, J.; Aberle, CM.; Gnanashanmugam, S. Innovative Approaches to Mitral Valve Repair and Replacement. In: Carabello BA. , editor. Valvular Heart Disease. London: Springer London; 2020. pp. 131–76. 88. Jouan J, Berrebi A, Chauvaud S, Menasche P, Carpentier A, Fabiani JN. Mitral valve reconstruction in Barlow disease: long-term echographic results and implications for surgical management. J Thorac Cardiovasc Surg. 2012;143:S17–20. https://doi.org/10.1016/j.jtcvs.2011.11.016. 89. Rausch MK, Zollner AM, Genet M, Baillargeon B, Bothe W, Kuhl E. A virtual sizing tool for mitral valve annuloplasty. Int J Numer Method Biomed Eng. 2017;33:e02788. https://doi.org/10.1002/cnm.2788. 90. Lindman, BR.; Melby, SJ.; Quader, N.; Sintek, MA.; Moon, MR. Comprehensive Assessment of Primary Mitral Valve Disease: Clinical Presentation, Diagnosis, Medical and Surgical Therapy. In: Carabello BA. , editor. Valvular Heart Disease. London: Springer London; 2020. pp. 103–24. 92. Vassileva CM, Boley T, Markwell S, Hazelrigg S. Meta-analysis of short-term and long-term survival following repair versus replacement for ischemic mitral regurgitation. Eur J Cardiothorac Surg. 2011;39:295–303. https://doi.org/10.1016/j.ejcts.2010.06.034. 93. Muller DWM, Farivar RS, Jansz P, et al. Transcatheter mitral valve replacement for patients with symptomatic mitral regurgitation: a global feasibility trial. J Am Coll Cardiol. 2017;69:381–91. https://doi.org/10.1016/j.jacc.2016.10.068. 94. Mirabel M, Iung B, Baron G, et al. What are the characteristics of patients with severe, symptomatic, mitral regurgitation who are denied surgery? Eur Heart J. 2007;28:1358–65. https://doi.org/10.1093/eurheartj/ehm001. 95. Kheradvar A, Groves EM, Simmons CA, et al. Emerging trends in heart valve engineering: part III. Novel technologies for mitral valve repair and replacement. Ann Biomed Eng. 2015;43:858–70. https://doi.org/10.1007/s10439-014-1129-y. 96. Nishimura RA, Otto CM, Bonow RO, et al. 2014 AHA/ACC guideline for the management of patients with valvular heart disease: a report of the American College of Cardiology/American Heart Association task force on practice guidelines. Circulation. 2014;129:e521–643. https://doi.org/10.1161/CIR.0000000000000031. 97. Praz F, Spargias K, Chrissoheris M, et al. Compassionate use of the pascal transcatheter mitral valve repair system for patients with severe mitral regurgitation: a multicentre, prospective, observational, first-in-man study. Lancet. 2017;390:773–80. https://doi.org/10.1016/S0140-6736(17)31600-8. 98. Maisano F, Taramasso M, Nickenig G, et al. Cardioband, a transcatheter surgical-like direct mitral valve annuloplasty system: early results of the feasibility trial. Eur Heart J. 2016;37:817–25. https://doi.org/10.1093/eurheartj/ehv603. 99. Testa L, Latib A, Montone RA, Bedogni F. Transcatheter mitral valve regurgitation treatment: state of the art and a glimpse to the future. J Thorac Cardiovasc Surg. 2016;152:319–27. https://doi.org/10.1016/j.jtcvs.2016.04.055. 100. Regueiro A, Granada JF, Dagenais F, Rodes-Cabau J. Transcatheter mitral valve replacement: insights from early clinical experience and future challenges. J Am Coll Cardiol. 2017;69:2175–92. https://doi.org/10.1016/j.jacc.2017.02.045. 102. Khan JM, Rogers T, Babaliaros VC, Fusari M, Greenbaum AB, Lederman RJ. Predicting left ventricular outflow tract obstruction despite anterior mitral leaflet resection: the “Skirt NeoLVOT”. JACC Cardiovasc Imaging. 2018;11:1356–9. https://doi.org/10.1016/j.jcmg.2018.04.005. 103. Babaliaros VC, Greenbaum AB, Khan JM, et al. Intentional percutaneous laceration of the anterior mitral leaflet to prevent outflow obstruction during transcatheter mitral valve replacement: first-in-human experience. JACC Cardiovasc Interv. 2017;10:798–809. https://doi.org/10.1016/j.jcin.2017.01.035. 104. Connell PS, Azimuddin AF, Kim SE, et al. Regurgitation hemodynamics alone cause mitral valve remodeling characteristic of clinical disease states in vitro. Ann Biomed Eng. 2016;44:954–67. https://doi.org/10.1007/s10439-015-1398-0. 107. Kunzelman KS, Cochran RP, Chuong C, Ring WS, Verrier ED, Eberhart RD. Finite element analysis of the mitral valve. J Heart Valve Dis. 1993;2:326–40. 108. Dahl SK, Vierendeels J, Degroote J, Annerel S, Hellevik LR, Skallerud B. FSI simulation of asymmetric mitral valve dynamics during diastolic filling. Comput Methods Biomech Biomed Engin. 2012;15:121–30. https://doi.org/10.1080/10255842.2010.517200. 111. Toma M, Einstein DR, Bloodworth CH 4th, Cochran RP, Yoganathan AP, Kunzelman KS. Fluid-structure interaction and structural analyses using a comprehensive mitral valve model with 3D chordal structure. Int J Numer Method Biomed Eng. 2017;33:e2815. https://doi.org/10.1002/cnm.2815. 112. Mao W, Caballero A, McKay R, Primiano C, Sun W. Fully-coupled fluid-structure interaction simulation of the aortic and mitral valves in a realistic 3D left ventricle model. PLoS One. 2017;12:e0184729. https://doi.org/10.1371/journal.pone.0184729. 113. Kunzelman KS, Reimink MS, Cochran RP. Flexible versus rigid ring annuloplasty for mitral valve annular dilatation: a finite element model. J Heart Valve Dis. 1998;7:108–16. 114. Maisano F, Redaelli A, Soncini M, Votta E, Arcobasso L, Alfieri O. An annular prosthesis for the treatment of functional mitral regurgitation: finite element model analysis of a dog bone-shaped ring prosthesis. Ann Thorac Surg. 2005;79:1268–75. https://doi.org/10.1016/j.athoracsur.2004.04.014. 117. Stevanella M, Maffessanti F, Conti CA, et al. Mitral valve patient-specific finite element modeling from cardiac MRI: application to an annuloplasty procedure. Cardiovasc Eng Technol. 2011;2:66–76. https://doi.org/10.1007/s13239-010-0032-4. 118. Choi A, Rim Y, Mun JS, Kim H. A novel finite element-based patient-specific mitral valve repair: virtual ring annuloplasty. Biomed Mater Eng. 2014;24:341–7. https://doi.org/10.3233/BME-130816. 119. Mansi T, Voigt I, Georgescu B, et al. An integrated framework for finite-element modeling of mitral valve biomechanics from medical images: application to MitralClip intervention planning. Med Image Anal. 2012;16:1330–46. https://doi.org/10.1016/j.media.2012.05.009. 121. Sturla F, Redaelli A, Puppini G, Onorati F, Faggian G, Votta E. Functional and biomechanical effects of the edge-to-edge repair in the setting of mitral regurgitation: consolidated knowledge and novel tools to gain insight into its percutaneous implementation. Cardiovasc Eng Technol. 2015;6:117–40. https://doi.org/10.1007/s13239-014-0208-4. 125. Chatterjee A, Bajaj NS, McMahon WS, et al. Transcatheter pulmonary valve implantation: a comprehensive systematic review and meta-analyses of observational studies. J Am Heart Assoc. 2017;6:e006432. https://doi.org/10.1161/JAHA.117.006432. 126. Malekzadeh-Milani S, Ladouceur M, Cohen S, Iserin L, Boudjemline Y. Results of transcatheter pulmonary valvulation in native or patched right ventricular outflow tracts. Arch Cardiovasc Dis. 2014;107:592–8. https://doi.org/10.1016/j.acvd.2014.07.045. 127. Meadows JJ, Moore PM, Berman DP, et al. Use and performance of the Melody transcatheter pulmonary valve in native and postsurgical, nonconduit right ventricular outflow tracts. Circ Cardiovasc Interv. 2014;7:374–80. https://doi.org/10.1161/CIRCINTERVENTIONS.114.001225. 128. Faccini A, Butera G. Tricuspid regurgitation as a complication of Edwards Sapien XT valve implantation in pulmonary position a problem to deal with. Catheter Cardiovasc Interv. 2018;91:927–31. https://doi.org/10.1002/ccd.27527. 129. Suzuki I, Shiraishi Y, Yabe S, et al. Engineering analysis of the effects of bulging sinuses in a newly designed pediatric pulmonary heart valve on hemodynamic function. J Artif Organs. 2012;15:49–56. https://doi.org/10.1007/s10047-011-0609-1. 130. Capelli C, Sauvage E, Giusti G, et al. Patient-specific simulations for planning treatment in congenital heart disease. Interface Focus. 2018;8:20170021. https://doi.org/10.1098/rsfs.2017.0021. 131. Li CL, Baird C, Yao J, et al. Computational modeling of human bicuspid pulmonary valve dynamic deformation in patients with tetralogy of fallot. Comput Model Eng Sci. 2019;119:227–44. https://doi.org/10.32604/cmes.2019.06036. 133. Schofer J, Bijuklic K, Tiburtius C, Hansen L, Groothuis A, Hahn RT. First-in-human transcatheter tricuspid valve repair in a patient with severely regurgitant tricuspid valve. J Am Coll Cardiol. 2015;65:1190–5. https://doi.org/10.1016/j.jacc.2015.01.025. 134. Latib A, Agricola E, Pozzoli A, et al. First-in-man implantation of a tricuspid annular remodeling device for functional tricuspid regurgitation. JACC Cardiovasc Interv. 2015;8:e211–14. https://doi.org/10.1016/j.jcin.2015.06.028. 136. Lapenna E, De Bonis M, Verzini A, et al. The clover technique for the treatment of complex tricuspid valve insufficiency: midterm clinical and echocardiographic results in 66 patients. Eur J Cardiothorac Surg. 2010;37:1297–303. https://doi.org/10.1016/j.ejcts.2009.12.020. 137. Vismara R, Gelpi G, Prabhu S, et al. Transcatheter edge-to-edge treatment of functional tricuspid regurgitation in an ex vivo pulsatile heart model. J Am Coll Cardiol. 2016;68:1024–33. https://doi.org/10.1016/j.jacc.2016.06.022. 138. Dabiri Y, Yao J, Sack KL, Kassab GS, Guccione JM. Tricuspid valve regurgitation decreases after MitraClip implantation: fluid structure interaction simulation. Mech Res Commun. 2019;97:96–100. https://doi.org/10.1016/j.mechrescom.2019.04.009. 141. Mummert J, Sirois E, Sun W. Quantification of biomechanical interaction of transcatheter aortic valve stent deployed in porcine and ovine hearts. Ann Biomed Eng. 2013;41:577–86. https://doi.org/10.1007/s10439-012-0694-1. 142. Qian Z, Wang K, Liu S, et al. Quantitative prediction of paravalvular leak in transcatheter aortic valve replacement based on tissue-mimicking 3D printing. JACC Cardiovasc Imaging. 2017;10:719–31. https://doi.org/10.1016/j.jcmg.2017.04.005. 143. Ripley B, Kelil T, Cheezum MK, et al. 3D printing based on cardiac CT assists anatomic visualization prior to transcatheter aortic valve replacement. J Cardiovasc Comput Tomogr. 2016;10:28–36. https://doi.org/10.1016/j.jcct.2015.12.004. 145. Tanaka Y, Saito S, Sasuga S, et al. Quantitative assessment of paravalvular leakage after transcatheter aortic valve replacement using a patient-specific pulsatile flow model. Int J Cardiol. 2018;258:313–20. https://doi.org/10.1016/j.ijcard.2017.11.106. 146. Hatoum H, Dollery J, Lilly SM, Crestanello J, Dasi LP. Impact of patient-specific morphologies on sinus flow stasis in transcatheter aortic valve replacement: an in vitro study. J Thorac Cardiovasc Surg. 2019;157:540–9. https://doi.org/10.1016/j.jtcvs.2018.05.086. 149. Morrison TM, Pathmanathan P, Adwan M, Margerrison E. Advancing regulatory science with computational modeling for medical devices at the FDA’s office of science and engineering laboratories. Front Med (Lausanne). 2018;5:241. https://doi.org/10.3389/fmed.2018.00241. 150. Chiu WC, Alemu Y, McLarty AJ, Einav S, Slepian MJ, Bluestein D. Ventricular assist device implantation configurations impact overall mechanical circulatory support system thrombogenic potential. ASAIO J. 2017;63:285–92. https://doi.org/10.1097/MAT.0000000000000488. |